Research Program
Our lab’s research goal is to determine the structure, function, and electron transfer mechanism of nanowires and to evaluate their role in bacterial respiration, communication, and infections. By combining experiments and computations , our team is addressing three key questions: (1) How do microbes build & use nanowires? (2) How are electrons transferred from the bacterial cytoplasm to surface-displayed nanowires? (3) Can nanowire conductivity be tuned using light, pressure, temperature, electromagnetic fields, and non-natural ‘click’ chemistry functionalities to control bacterial behavior? To achieve these goals, we have made the following contributions:
1. Discovery of protein nanowires and their structures, functions, assembly, and charging mechanisms.To understand how microbes use nanowires, first, our lab developed a method to grow cells under nanowire-producing conditions. Then, our team purified the nanowires to obtain high-quality cryo-EM data essential to determine the nanowire structure and function. As the initial nanowire structure obtained by ourlab did not correspond to Type-4 pili (T4P), we collaborated with F. Wang and E. Egelman (U. Virginia), who reanalyzed our data and determined the atomic structure of nanowires. We were surprised to find an unprecedented polymer of multi-heme cytochrome OmcS. Our lab showed that the closely-stacked (<4-6 Å) hemes in OmcS polymers allow for the conductivity of these purified nanowires to be identical to that measured previously for Geobacter (Cell‘19). These results directly demonstrate that nanowires are polymers of cytochromes . Our high-resolution cryo-EM structure enabled for the first time cryo-EM protein ‘sequencing’, whereby difficult-to-purify proteins can be identified using a combination of cryo-EM and mass spectrometry. Many are using this bottom-up structural proteomics method, which has > 400 citations in 5 years. Our lab subsequently discovered that to send electrons distances greater than 100 times the microbe’s size in biofilms, Geobacter biofilms produce another nanowire type made up of cytochrome OmcZ, which is 1000-fold more conductive than OmcS (Nature Chem. Bio.‘20). We developed a new purification method to obtain the highest-resolution OmcZ structure (Nature Micro.‘23). We also achieved the first synthetic assembly of nanowires at a high yield. This work suggested that cells assemble nanowires on demand using a protease connected to omcZ via a small RNA. We are now collaborating with Steitz lab (MB&B) to study the structure and function of that RNA. We also found that OmcZ homologs are expressed by large plasmids and giant (1 Mb) extrachromosomal DNA elements called Borgs produced by Methanoperedens archaea that control global methane levels. With archaea samples from Banfield (UC Berkeley), Wegener (Max Plank), and Welte (Netherlands), our lab is now determining the structures and functions of these OmcZ-like filaments to test if nanowires control microbial methane production.
2. Discovery of intracellular pili involved in nanowire secretion & engineering of pili to be nanowires. Our lab’s structural, functional, and cellular localization studies suggested that G. sulfurreducens pili cannot be nanowires but instead function as endopilus-like pistons to secrete cytochrome nanowires (Nature, 2021). Although soil bacterial pili are intracellular, our lab has found that other bacteria can use surface-displayed pili for electron export to switch to fast-growing metabolism. This is owing to highly conserved aromatic residues serving as an electron escape route to avoid oxidative damage . Combining computations by Batista (Yale) with my lab’s measurements, we showed that this electron transfer is due to a novel proton-rocking mechanism, whereby energetics of the proton acceptor and proximity to aromatics control protein conductivity. Most publications report protein conductivities that include high contact resistances, which dominate the results. Our lab’s 4-probe method enabled the first measurement of intrinsic (contact-free) protein conductivity. We showed that proteins conduct electrons at physiological potentials without metal cofactors (PNAS‘20). Proteins were previously considered electron non-conductors. My lab’s work can potentially revolutionize the field of protein electronics. For example, with Isaacs (MCDB), we increased the electron conductivity of E. coli pili by 100-fold with controlled filament assembly using non-natural, ‘click’ chemistry functionality (Nature Comm.‘22)
3. Tuning nanowire conductivity to control microbial functions using Electromagnetic fields, light, and temperature. Our lab uses nanowires to image and control bacterial growth with electric (E)-fields, building on our discovery that microbes produce OmcZ nanowires by exploiting the natural redox potential difference (~0.1 V) at the electron donor/acceptor interface (Nature Chem. Bio.‘20). This voltage generates large electric fields across a typical cell membrane of 100 nm (0.1V/100 nm=106 V/m). These E-fields cause overexpression of nanowire genes to modulate microbial metabolism, communication, and biofilm formation. Similar to E-fields, magnetic (B) fields generated by minerals in the soil double the growth rate of G. sulfurreducens by altering the redox potential of cytochromes. Our measurements showed that these minerals allow the sediments to transport electrons over centimeters. They can also convert incident light into electricity. This enables G. sulfurreducens to grow 8-times faster upon illumination, despite not being photosynthetic, and results in increased growth rate and substrate consumption. Our lab showed that nanowire conductivity increases by up to 30,000-fold by light, pressure, temperature, electromagnetic fields, which could accelerate microbial growth. Ion-conducting protein channels enable remote monitoring and control of neuronal signaling by opto-, electro-, magneto-, and mechanogenetics. Using these probes, microbial nanowires can be exploited to monitor and control diverse environmentally- and clinically-important phenomena, such as carbon and mineral cycling, bioremediation, corrosion, chemical and biofuel production as well as infections and dysbiosis caused by fast-growing pathogens due to their access to electron acceptors.
Ongoing Research: We are capitalize on the above insights we accumulated to develop in situ metabolic, multifunctional, and structural imaging of nanowire-producing cells. Combining imaging with multi-omics modeling, we will identify the mechanisms of nanowire assembly, redox regulation, conductivity, and protein-protein interactions that wire the inside of the cell to the outside. Our approach is applicable to diverse microbes. Using what we learn from these studies, our long-term vision is to monitor and control the growth of microbes residing in the deep ocean, in soil, or the human body to advance the understanding of nanowires in four areas:
1) Fundamental studies to elucidate how diverse microbes assemble and use various nanowires.
2) Repair environmental health by using microbial communities integrated through nanowire-mediated electron exchange;
3) Restore rhizosphere health by identifying and targeting nanowire-mediated microbe-plant interactions; and
4) Restore human health by controlling the growth and colonization of clinically important microbes by targeting nanowire-mediated metabolic electron transfer.
New Method to Visualize Electron Transfer in Bacterial Protein Nanowires
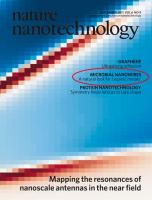
Energy and Environmental Science 5, 5790-5797.
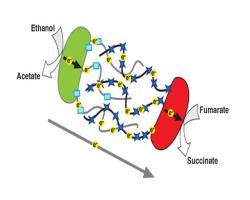
Bacteria use metalloproteins as humans use their lungs.
We have demonstrated that a high-performance supercapacitor can be synthesized using electron transport and storage properties of living microbial biofilms. ChemPhysChem.13, 463 – 468.